Contributing Writer
- FMA
- The Fabricator
- FABTECH
- Canadian Metalworking
Categories
- Additive Manufacturing
- Aluminum Welding
- Arc Welding
- Assembly and Joining
- Automation and Robotics
- Bending and Forming
- Consumables
- Cutting and Weld Prep
- Electric Vehicles
- En Español
- Finishing
- Hydroforming
- Laser Cutting
- Laser Welding
- Machining
- Manufacturing Software
- Materials Handling
- Metals/Materials
- Oxyfuel Cutting
- Plasma Cutting
- Power Tools
- Punching and Other Holemaking
- Roll Forming
- Safety
- Sawing
- Shearing
- Shop Management
- Testing and Measuring
- Tube and Pipe Fabrication
- Tube and Pipe Production
- Waterjet Cutting
Industry Directory
Webcasts
Podcasts
FAB 40
Advertise
Subscribe
Account Login
Search
Plasma cutting stainless steel and aluminum
Investigating thermal and chemical changes in the heat-affected zone
- By Charles M. Hackett
- July 12, 2001
- Article
- Plasma Cutting
The plasma cutting process may be used to cut any conductive material, including carbon steels, stainless steels, aluminum, copper, brass, cast metals, and exotic alloys. Each of these materials behaves differently when subjected to the intense heating and cooling of the plasma cutting process.
Mild steel is the most commonly used material in metal fabrication. However, the corrosion resistance, high strength-to-weight ratio, thermal properties, and aesthetics of stainless steel and aluminum alloys make these materials attractive for many applications. The weldability and other material characteristics of plasma-cut carbon steels have been well-documented. A recent study by engineers at the author's company (published in the September 2000 issue of The FABRICATOR®, pages 28-31) characterized the heat-affected zone (HAZ) for carbon steels and suggested several plasma process alternatives to minimize HAZ.
In a continuation of that study, scientists have further investigated the material properties of stainless steels and aluminum. The two goals of the study were to:
- Characterize thermal and chemical changes in plasma-cut stainless steel and aluminum alloys.
- Recommend process alternatives that may improve aesthetics and cut quality to improve forming and fabricating of these materials.
The Experiment
Three plasma arc cutting (PAC) systems were used to prepare cut samples for metallographic analysis:
- Water-injection PAC (WIP). This process uses a plasma-forming gas and injection water. Injection water impinges directly onto the plasma jet to constrict the arc and protect the torch nozzle. For this experiment, a nitrogen plasma water-injection system was used.
- Conventional Dual-gas (CDG) PAC. This process uses a plasma-forming gas and a shield gas that cools the front end of the torch and assists in cut quality. For this experiment, several plasma and shield gas combinations were used: air plasma-air shield, nitrogen plasma-nitrogen shield, nitrogen plasma-carbon dioxide shield, and argon/hydrogen plasma-nitrogen shield.
- High-precision PAC (HPP). This process uses a strong vortex of plasma gas and special consumable geometries to achieve greater arc constriction and higher energy density. For this experiment, air plasma-air methane shield and argon/hydrogen plasma-nitrogen shield were used in a high-precision system.
Cut samples ranged in thickness from 1 to 25 mm. Figure 1 summarizes the operating conditions.
Researchers removed a small section from each cut sample (see Figure 2). The section was placed in a metallographic mount, polished, and electrochemically etched to reveal details in the microstructure. The term microstructure refers to the microscopic grain structure of a material, which determines many of its physical properties.
Measurements and analysis of each cut sample were made using optical microscopy. Two magnifications were used: 100, which permits measurement of HAZ features, and 400 to 500, which allow analysis of HAZ phase content.
The Materials Used
Most of the samples were cut from sheets of 304 SS. Because 300 series stainless has an austenitic phase structure, a limited number of martensitic 410 SS samples also were analyzed for comparison. The significant difference between 300 and 400 series is the nickel and iron content: 410 SS has higher iron content (84 to 86 percent) and no nickel, while 304 SS has significant nickel content (8 to 10.5 percent).
Because of their different chemical compositions, 300 series and 400 series materials also have different thermophysical properties, such as thermal conductivity and specific heat capacity, that affect the behavior of the metal during cutting and the resulting HAZ. For the aluminum cutting, alloy grade 6061 (nominal composition: 1 percent magnesium, 0.6 percent silicon, 0.2 percent chromium, 0.3 percent copper, balance aluminum) was used.
Stainless Steel Cut Edges
The appearance of the cut edge varies considerably with PAC process and gas selection (see Figure 3). Air plasma or oxidizing shield gas, such as air or CO2, tends to produce a dark, oxidized cut edge.
Nitrogen plasma, argon/hydrogen (H35) plasma, or reducing shield gas (methane or other hydrogen-containing gases) tends to react chemically with oxygen present in the kerf, permitting little or no oxide to form on the cut edge. The plasma and shield gases, consumable design, and condition of the consumables influence other characteristics of the cut edge, such as roughness, cut angle, and dross.
A resolidified layer along the cut edge characterizes the HAZ in austeniticstainless steelcuts (such as alloy 304). This layer is evident in the metallographic cross section in Figures 4a (low magnification) and 4b (high magnification) for a 400-amp nitrogen plasma cut in 12.8-mm-thick 304 SS. This material has melted during the cutting process, adhered to the sides of the cut, and resolidified.
Although the phase composition of this layer is not clear, its fine-grained microstructure shows some evidence of selective etching between grains, indicating precipitates may have formed along grain boundaries.
All austenitic stainless steel cut specimens investigated in this study behaved similarly. Comparing Figure 4c, a high-magnification photomicrograph of a 200-amp CDG air plasma cut in 12.8-mm-thick 304 SS, and Figure 4d (a similar view for 200-amp argon/hydrogen plasma) to Figure 4b reveals that each has a fine-grained, resolidified layer on top of the original core metal.
The thermal conductivity of 300 series is relatively low, which causes a concentration of thermal effects at the cut edge. Because the austenitic phase transformation occurs at relatively high temperatures, no significant solid-state phase transformation in austenitic PAC edges is evident.
Shown in Figures 4e and 4f are low-magnification micrographs of austenitic (316) and martensitic (410) alloys cut with identical cutting processes (120-amp CDG nitrogen plasma) in 3.3-mm-thick material. At this magnification, hardly any of the resolidified layer is visible in either cut sample.
While the 316 SS microstructure is relatively unchanged from surface to core, the 410 SS microstructure shows clear evidence of a solid-state phase transformation. Ferritic and martensitic stainless steel alloys behave much like carbon steel in that a solid-state phase transformation normally is found in the HAZ.
Analysis of the cut specimens indicates that the resolidified layer typically is about 10 to 30 m thick, though some thick stainless steel specimens did have measurements three to six times larger.
- Images (a) and (b) show a 304 SS alloy specimen, 12.8 mm thick, cut with 400-amp WIP. Image(a)is 100x magnification; image (b) is 500x magnification.
- Image (c) and (d) show a specimen of 304 SS, 12.8 mm thick, cut with 200-amp CDG, at 500x magnification. Image (c) was made with air plasma; image (d) was made with argon/hydrogen plasma.
- Image (e) is 316 SS; image (f) is martensitic 410 SS; both are 100x magnification. Both are 3.3-mm-thick specimens cut with CDG nitrogen plasma at 120 amps. Note the presence of a phase change in the HAZ in the martensitic specimen.
- Images (g) and (h) are 500x magnifications of 3.3-mm-thick 304 SS edges cut with CDG nitrogen plasma. Specimen (g) has a higher sulfur content in the bulk material than specimen (h). The resolidified layer in (g) is about one-half the thickness of that in (h).
The thickness of the resolidified layer in PAC austenitic stainless steel most likely is related to chemical composition of the SS, as well as to the particular PAC process used to make a cut. For example, Figures 4g and h show high-magnification photomicrographs of cut specimens made with 120-amp CDG nitrogen plasma.
The 3.3-mm 304 SS material was secured from two different sources. Both samples (Figures 4g and 4h) have a thin oxide layer that lies on top of a resolidified layer. The resolidified layer in sample 4h is approximately twice as thick as that in sample 4g.
Although several slight differences in composition are apparent, the most significant may be that of sulfur. Sulfur is a surface-active element that can significantly change the behavior of molten metal in high-temperature processing of metals (for example, welding). The increased presence of sulfur in sample 4h may have led to the thinner resolidified layer.
In addition to the microstructural evaluations described here, microhardness measurements were made near the cut edge and in the core of the 304 and 316 SS samples. Though edge hardness generally was slightly higher than that of the core material, the average change in hardness often was within the variation of the multiple measurements made.
While these results indicate that PAC does not substantially harden austenitic stainless steel edges, one might expect different results for ferritic and martensitic stainless steel because of the likelihood of solid-state phase transformations. No measurements have been made on these materials, however.
The weldability of stainless steel PAC edges is a subject reserved for future study. Generally speaking, however, the surface oxides formed on stainless steel cut with oxidizing plasma or shield gas (such as air or CO2) need to be removed by grinding before good welds can be made.
PAC processes that use a reducing plasma or shield gas, such as argon/ hydrogen or methane, produce cut edges with substantially less surface oxide that require little or no preparation before welding.
Aluminum Cut Edges
The appearance of the aluminum cut edge also varies with PAC process and gas selection (see Figure 5). The surface condition of aluminum edges frequently is characterized by relative roughness, thin oxide layers, intergranular cracks, and porosity near the cut edge surface.
The HAZ of aluminum alloy cut specimens analyzed in this study has both a resolidified layer, as well as a solid-state phase transformation. However, the phase structure of the aluminum alloy often is only faintly visible in the metallographic micrographs.
For example, the low-magnification (see Figure 6a) and high-magnification (see Figure 6b) cross-section images of 6.3-mm-thick aluminum alloy cut with 70-amp air plasma and methane shield reveal a rough cut edge with some porosity present.
The extent of the HAZ (including both the resolidified and phase-transformed layers) generally is indicated by the presence of grain boundary silicide precipitates. The microstructure of cut specimens prepared with CDG air plasma (see Figure 6c) and CDG argon/hydrogen plasma (see Figure 6d) is qualitatively similar to that in Figures 6a and 6b.
Because of the high thermal conductivity of aluminum alloys, the overall thickness of the HAZ may be significant. The resolidified layer often is as thick as the region defined by the phase transformation. The thickness of the HAZ is related to process conditions, such as cut speed and process gas, as well as material thickness. The width of the HAZ is greater for thicker material that is cut at lower speed.
Microhardness measurements of aluminum alloy 6061 near the cut edge and in the cut sample core region indicate that hardness is decreased significantly in the HAZ. The thermal cycle of heating and cooling that occurs during the PAC process essentially is an annealing process. This thermal cycle degrades the heat treat of the metal (usually T6) and returns it to an annealed state.
The weldability of aluminum alloy cut edges made with PAC is best when surface oxides are not present. If oxidizing process gases are used in the plasma or shield, the edges may need to be ground before welding. Weldability of edges cut with reducing plasma or shield likely will be better. Because aluminum reacts so readily with oxygen, grinding still may be required to prepare the cut edge.
Summary of Results
300 Series SS. Plasma arc cutting of austenitic stainless steel alloys produces a HAZ characterized by a thin layer of resolidified metal adhered to the cut edge. Little or no solid-state phase transformation is observed in the microstructure.
The thickness of the resolidified layer generally is in the 10- to 30-m range (less than 0.001 inch). The formation of this layer may be influenced by the presence of trace elements (such as sulfur) in the original metal.
Little difference in microhardness is present at the cut edge compared with that of the core material.
400 Series SS. Martensitic and ferritic stainless steels behave differently from 300-series alloys. Plasma-cut 410 series stainless steels show evidence of solid-state phase transformation along the HAZ.
Alloy 6061 Aluminum. Cut edges have a HAZ characterized by both solid-state phase transformation and a resolidified layer. The surfaces of aluminum alloy cut edges are rough and may have intergranular cracks and porosity. The presence of surface oxides may affect weldability of both stainless steel and aluminum alloy materials.
All these stainless steel and aluminum materials readily form surface oxides that may affect weldability.
HAZ Findings
This study of thermal and chemical effects in plasma-cut stainless and aluminum confirmed many of the findings of previous studies on carbon steel.
- The HAZ is small in plasma-cut pieces. Most HAZ measurements in this study were less than 0.001 inch thick.
- HAZ varies with speed and power. The extent of the HAZ in mild steel is related to process variables, such as cut speed and power, as well as material thickness.
- Faster cutting produces less HAZ. Decreasing the time required to perform a cut by using high amperage and high-speed conditions reduces the HAZ.
- More heat (per square inch) can produce less HAZ. High-energy density processes (more power per unit area), such as high-precision PAC, produce less HAZ.
For some applications the HAZ must be removed mechanically before welding to prevent embrittlement and weld failure, but the HAZ of plasma-cut stainless and aluminum generally is small and can be further minimized through process controls.
About the Author
subscribe now
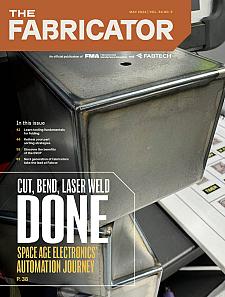
The Fabricator is North America's leading magazine for the metal forming and fabricating industry. The magazine delivers the news, technical articles, and case histories that enable fabricators to do their jobs more efficiently. The Fabricator has served the industry since 1970.
start your free subscription- Stay connected from anywhere
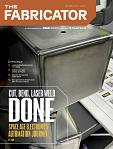
Easily access valuable industry resources now with full access to the digital edition of The Fabricator.
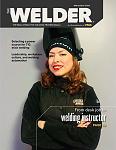
Easily access valuable industry resources now with full access to the digital edition of The Welder.
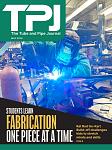
Easily access valuable industry resources now with full access to the digital edition of The Tube and Pipe Journal.
- Podcasting
- Podcast:
- The Fabricator Podcast
- Published:
- 04/16/2024
- Running Time:
- 63:29
In this episode of The Fabricator Podcast, Caleb Chamberlain, co-founder and CEO of OSH Cut, discusses his company’s...
- Trending Articles
Tips for creating sheet metal tubes with perforations
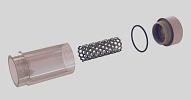
Supporting the metal fabricating industry through FMA
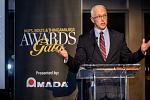
JM Steel triples capacity for solar energy projects at Pennsylvania facility
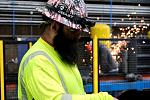
Are two heads better than one in fiber laser cutting?
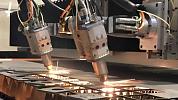
Fabricating favorite childhood memories
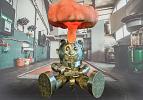
- Industry Events
16th Annual Safety Conference
- April 30 - May 1, 2024
- Elgin,
Pipe and Tube Conference
- May 21 - 22, 2024
- Omaha, NE
World-Class Roll Forming Workshop
- June 5 - 6, 2024
- Louisville, KY
Advanced Laser Application Workshop
- June 25 - 27, 2024
- Novi, MI